A novel technique that nudges single atoms to switch places within an atomically thin material could bring scientists another step closer to realizing theoretical physicist Richard Feynman’s vision of building tiny machines from the atom up.
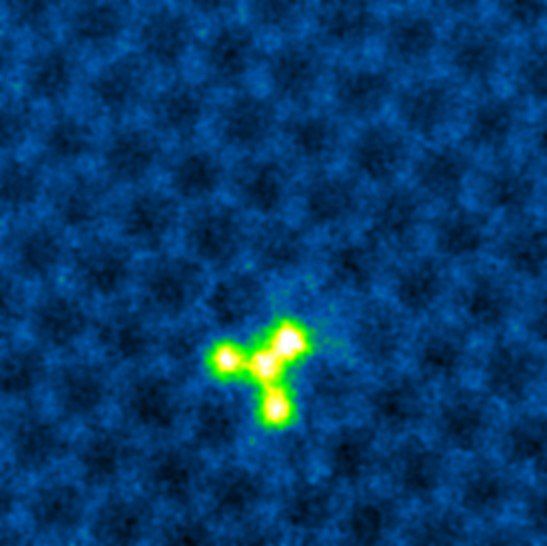
A novel technique that nudges single atoms to switch places within an atomically thin material could bring scientists another step closer to realizing theoretical physicist Richard Feynman’s vision of building tiny machines from the atom up.
Six years ago, the Voyager 1 spacecraft informed scientists that it had become the first man-made object to enter interstellar space. Now, Voyager 2 has begun to return signs that its own exit from the Solar System could be coming soon.
Two of Voyager 2’s instruments have measured an increase in the number of high-energy particles called cosmic rays hitting the spacecraft, according to a NASA release. Scientists think that the heliosphere, the region of particles and magnetic fields under the Sun’s influence, blocks some cosmic rays. An increase in their rate means that the probe could be nearing the heliopause, the heliosphere’s outer boundary.
For the first time, an international team of researchers has used a quantum computer to create artificial life—a simulation of living organisms that scientists can use to understand life at the level of whole populations all the way down to cellular interactions.
With the quantum computer, individual living organisms represented at a microscopic level with superconducting qubits were made to “mate,” interact with their environment, and “die” to model some of the major factors that influence evolution.
The new research, published in Scientific Reports on Thursday, is a breakthrough that may eventually help answer the question of whether the origin of life can be explained by quantum mechanics, a theory of physics that describes the universe in terms of the interactions between subatomic particles.
There, engineers are doing something strange. They’re freezing computer chips to 460 degrees Fahrenheit below zero, colder than deep space, to simulate the quantum structure of the universe.
At such extreme temperatures these remarkable chips, called qubits, enable scientists to peer into the complex, uncertain interaction of particles at the atomic level — an unseen world in which seemingly contradictory results can exist simultaneously, a place where simply observing an interaction can change it. Or wreck it altogether.
“Quantum — it’s something weird,” said Mike Mayberry, Intel’s chief technology officer and general manager of Intel Labs.
Since the 1970s, astronomers and physicists have been gathering evidence for the presence in the universe of dark matter: a mysterious substance that manifests itself through its gravitational pull. However, despite much effort, none of the new particles proposed to explain dark matter have been discovered. In a review that was published in Nature this week, physicists Gianfranco Bertone (UvA) and Tim Tait (UvA and UC Irvine) argue that the time has come to broaden and diversify the experimental effort, and to incorporate astronomical surveys and gravitational wave observations in the quest for the nature of dark matter.
Over the past three decades, the search for dark matter has focused mostly on a class of particle candidates known as weakly interacting massive particles (or WIMPs). WIMPs appeared for a long time as a perfect dark matter candidate as they would be produced in the right amount in the early universe to explain dark matter, while at the same time they might alleviate some of the most fundamental problems in the physics of elementary particles, such as the large discrepancy between the energy scale of weak interactions and that of gravitational interactions.
Humanity is producing so much data every single minute that we either need to slow down, or scientists need to crack the problem of finding better ways of storing that data ASAP. Now, new research has taken us one step closer to the ultimate in compact data storage: putting data on a single atom.
As the basic building blocks of all matter, atoms are the smallest object we could possibly store a bit (a 1 or a 0) on, potentially shrinking down the size of existing hard drives by about a thousand times or so, if we can figure out how to get it to work.
Scientists have already made progress in storing bits on atoms, but only on a small scale and in tightly controlled lab conditions, which usually means extremely cold setups.
The Large Hadron Collider, a massive particle accelerator near Geneva, Switzerland, just discovered two new baryons and hints of a meson.
Absolutely terrifying.
The views expressed in the contents above are those of our users and do not necessarily reflect the views of MailOnline.
There’s something out there that physicists have never seen before, and it’s coming up from the bottom of the Earth. Scientists think it’s a brand-new particle.
The building blocks of matter in our universe were formed in the first 10 microseconds of its existence, according to the currently accepted scientific picture. After the Big Bang about 13.7 billion years ago, matter consisted mainly of quarks and gluons, two types of elementary particles whose interactions are governed by quantum chromodynamics (QCD), the theory of strong interaction. In the early universe, these particles moved nearly freely in a quark-gluon plasma. Then, in a phase transition, they combined and formed hadrons, among them the building blocks of atomic nuclei, protons and neutrons.
In the current issue of Nature, an international team of scientists has presented an analysis of a series of experiments at major particle accelerators that sheds light on the nature of this transition. The scientists determined with precision the transition temperature and obtained new insights into the mechanism of cooling and freeze-out of the quark-gluon plasma into the current constituents of matter such as protons, neutrons and atomic nuclei. The team of researchers consists of scientists from the GSI Helmholtzzentrum für Schwerionenforschung in Darmstadt, and from the universities of Heidelberg and Münster (Germany), and Wroclaw (Poland).
A central result: The record-breaking high-energy experiments with the ALICE detector at the Large Hadron Collider (LHC) at the research center CERN produced matter in which particles and antiparticles coexisted in equal amounts, similar to the conditions in the early universe. The team has confirmed via analysis of the experimental data theoretical predictions that the phase transition between quark-gluon plasma and hadronic matter takes place at the temperature of 156 MeV, 120,000 times higher than that in the interior of the sun.