How did early black holes grow so quickly? A new study sheds some light on that by observing matter entering a black hole at 30% of the speed of light.
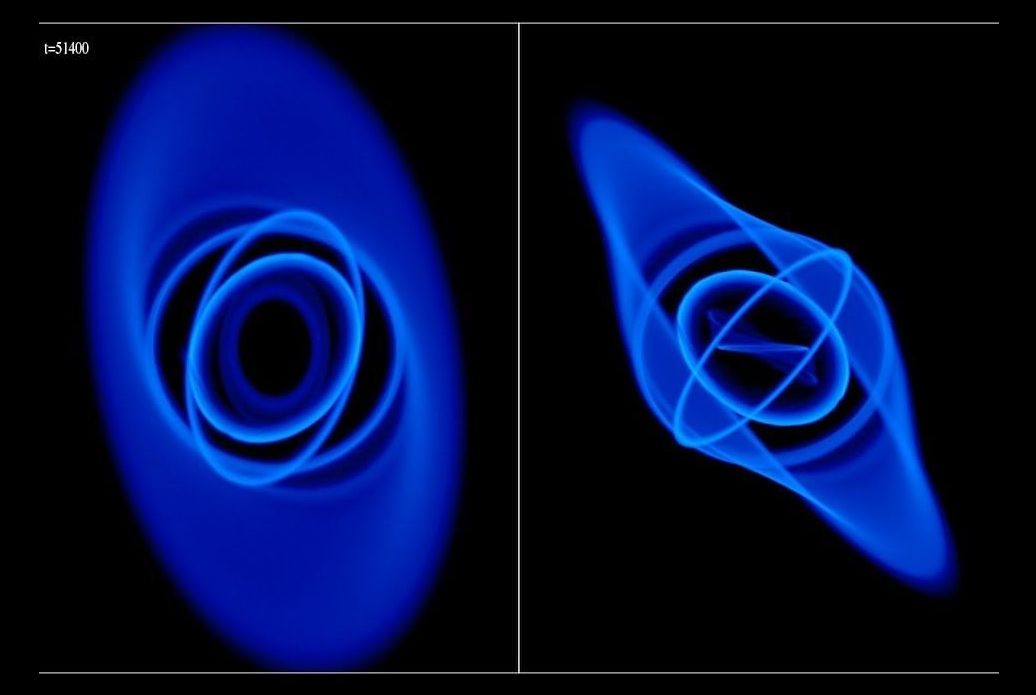
How did early black holes grow so quickly? A new study sheds some light on that by observing matter entering a black hole at 30% of the speed of light.
The building blocks of matter in our universe were formed in the first 10 microseconds of its existence, according to the currently accepted scientific picture. After the Big Bang about 13.7 billion years ago, matter consisted mainly of quarks and gluons, two types of elementary particles whose interactions are governed by quantum chromodynamics (QCD), the theory of strong interaction. In the early universe, these particles moved nearly freely in a quark-gluon plasma. Then, in a phase transition, they combined and formed hadrons, among them the building blocks of atomic nuclei, protons and neutrons.
In the current issue of Nature, an international team of scientists has presented an analysis of a series of experiments at major particle accelerators that sheds light on the nature of this transition. The scientists determined with precision the transition temperature and obtained new insights into the mechanism of cooling and freeze-out of the quark-gluon plasma into the current constituents of matter such as protons, neutrons and atomic nuclei. The team of researchers consists of scientists from the GSI Helmholtzzentrum für Schwerionenforschung in Darmstadt, and from the universities of Heidelberg and Münster (Germany), and Wroclaw (Poland).
A central result: The record-breaking high-energy experiments with the ALICE detector at the Large Hadron Collider (LHC) at the research center CERN produced matter in which particles and antiparticles coexisted in equal amounts, similar to the conditions in the early universe. The team has confirmed via analysis of the experimental data theoretical predictions that the phase transition between quark-gluon plasma and hadronic matter takes place at the temperature of 156 MeV, 120,000 times higher than that in the interior of the sun.
Space is filled with bizarre signals that we scramble to put meaning to — and now, researchers have detected yet another mysterious signal. This one emanated from near a neutron star, and for the first time, it’s infrared.
So, what’s nearby that could have created the weird signal? Scientists have a few ideas.
When a star reaches the end of its life, it typically undergoes a supernova explosion— the star collapses, and if it has enough mass, it will form a black hole. But if the star isn’t massive enough, it will form a neutron star. [Supernova Photos: Great Images of Star Explosions].
Researchers from Indiana University and the California Institute of Technology say the new simulations could help us better understand major astrophysical phenomena, such as gravitational waves.
‘The strength of the neutron star crust, especially the bottom of the crust, is relevant to a large number of astrophysics problems, but isn’t well understood,’ says Matthew Caplan, a postdoctoral research fellow at McGill University.
Neutron stars are the product of supernovas, and are extremely dense.
How do you know where anything is in space? Sure, you can say, “Oh, that star, it’s the one in the middle of the Big Dipper,” but that’s not very useful in an era of incredible telescopes peeping at galaxies billions of light-years away. On January 1, 2019, scientists will adopt the newest, internationally standardized frame of reference to help locate things in space.
The third edition of the International Celestial Reference Frame, or ICRF-3, is the most up-to-date version of the International Astronomy Union’s standardized reference frame. Imagine the universe as a graph from geometry—scientists need a place to put the origin and axes.
Machine learning supports 20-year-old theory of bizarre electron behaviour in high-temperature superconductor.
All the evidence shows our universe emerged from a single event: an eruption commonly known as the Big Bang.
What preceded that point is a mystery.
But it has significant implications.
It’s about the fate of our universe.
We know space is expanding. We can see that in the way all the galaxies around us are moving outward. But how far can it extend? What happens next?
It’s not easy being a “theory of everything.” A TOE has the very tough job of fitting gravity into the quantum laws of nature in such a way that, on large scales, gravity looks like curves in the fabric of space-time, as Albert Einstein described in his general theory of relativity. Somehow, space-time curvature emerges as the collective effect of quantized units of gravitational energy — particles known as gravitons. But naive attempts to calculate how gravitons interact result in nonsensical infinities, indicating the need for a deeper understanding of gravity.
String theory (or, more technically, M-theory) is often described as the leading candidate for the theory of everything in our universe. But there’s no empirical evidence for it, or for any alternative ideas about how gravity might unify with the rest of the fundamental forces. Why, then, is string/M-theory given the edge over the others?
The theory famously posits that gravitons, as well as electrons, photons and everything else, are not point-particles but rather imperceptibly tiny ribbons of energy, or “strings,” that vibrate in different ways. Interest in string theory soared in the mid-1980s, when physicists realized that it gave mathematically consistent descriptions of quantized gravity. But the five known versions of string theory were all “perturbative,” meaning they broke down in some regimes. Theorists could calculate what happens when two graviton strings collide at high energies, but not when there’s a confluence of gravitons extreme enough to form a black hole.
Machine-learning algorithms are helping to unravel the quantum behaviour of a type of superconductor that has baffled physicists for decades.
Researchers used artificial intelligence to spot hidden order in images of a bizarre state in high-temperature superconductors.
In the over two-decades-long search for dark matter, scientists so far have come up short. In recent years though, construction of new experiments and upgrades to already existing detectors are giving new hope that we’re closer than ever to understanding dark matter.
One of those new efforts is SABRE, an international collaboration that will house multiple detectors working in tandem in the southern and northern hemispheres: two at Italy’s Gran Sasso National Laboratory, and another at an underground lab in an Australian gold mine.
In episode two of The Most Unknown, Motherboard travels to Gran Sasso National Laboratory with physicist Davide D’Angelo and geomicrobiologist Jennifer Macalady to get an early look at SABRE’s latest phase of development.
Read more about The Most Unknown here: https://vice.video/2CQcMZw
Follow MOTHERBOARD
Facebook: http://www.facebook.com/motherboardtv
Twitter: http://twitter.com/motherboard
Tumblr: http://motherboardtv.tumblr.com/
Instagram: http://instagram.com/motherboardvice
More videos from the VICE network: https://www.fb.com/vicevideo